Preparation of cells for assessing ultrastructural localization of nanoparticles with transmission electron microscopy
We describe the use of transmission electron microscopy (TEM) for cellular ultrastructural examination of nanoparticle (NP)-exposed biomaterials. Preparation and imaging of electron-transparent thin cell sections with TEM provides excellent spatial resolution ( ∼ 1 nm), which is required to track these elusive materials. This protocol provides a step-by-step method for the mass-basis dosing of cultured cells with NPs, and the process of fixing, dehydrating, staining, resin embedding, ultramicrotome sectioning and subsequently visualizing NP uptake and translocation to specific intracellular locations with TEM. In order to avoid potential artifacts, some technical challenges are addressed. Based on our results, this procedure can be used to elucidate the intracellular fate of NPs, facilitating the development of biosensors and therapeutics, and provide a critical component for understanding NP toxicity. This protocol takes ∼ 1 week.
This is a preview of subscription content, access via your institution
Access options
Subscribe to this journal
Receive 12 print issues and online access
265,23 € per year
only 22,10 € per issue
Buy this article
- Purchase on SpringerLink
- Instant access to full article PDF
Prices may be subject to local taxes which are calculated during checkout
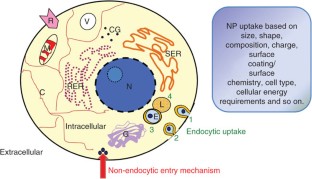
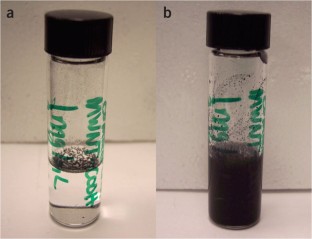
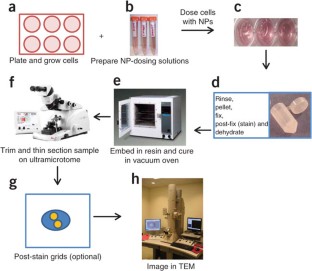

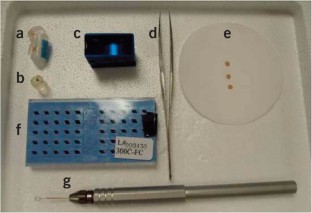
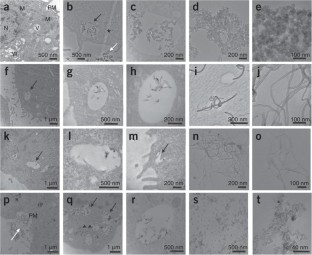
Similar content being viewed by others
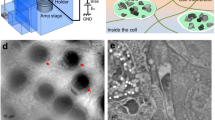
Nanoscale observation of PM2.5 incorporated into mammalian cells using scanning electron-assisted dielectric microscope
Article Open access 08 January 2021
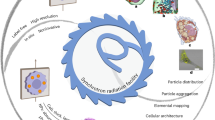
In situ label-free X-ray imaging for visualizing the localization of nanomedicines and subcellular architecture in intact single cells
Article 13 November 2023
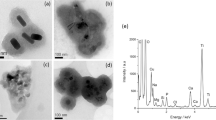
Nanoparticle corona artefacts derived from specimen preparation of particle suspensions
Article Open access 24 March 2020
References
- Colvin, V.L. The potential environmental impact of engineered nanomaterials. Nat. Biotechnol.21, 1166 (2003). ArticleCASPubMedGoogle Scholar
- Hoet, P.H., Nemmar, A. & Nemery, B. Health impact of nanomaterials? Nat. Biotechnol.22, 19 (2004). ArticleCASPubMedGoogle Scholar
- Maynard, A.D. et al. Safe handling of nanotechnology. Nature444, 267 (2006). ArticleCASPubMedGoogle Scholar
- Nel, A., Xia, T., Madler, L. & Li, N. Toxic potential of materials at the nanolevel. Science311, 622 (2006). ArticleCASPubMedGoogle Scholar
- Hussain, S.M., Hess, K.L., Gearhart, J.M., Geiss, K.T. & Schlager, J.J. In vitro toxicity of nanoparticles in BRL 3A rat liver cells. Toxicol. In Vitro19, 975–983 (2005). ArticleCASPubMedGoogle Scholar
- Braydich-Stolle, L., Hussain, S., Schlager, J.J. & Hofmann, M.-C. In vitro cytotoxicity of nanoparticles in mammalian germ-line stem cells. Tox. Sci.88, 412–419 (2005). ArticleCASGoogle Scholar
- Hussain, S. et al. The interaction of manganese nanotubes with PC-12 cells induces dopamine depletion. J. Tox. Sci.92, 456–463 (2006). ArticleCASGoogle Scholar
- Skebo, J.E., Grabinski, C.M., Schrand, A.M., Schlager, J.J. & Hussain, S.M. Assessment of metal nanoparticle agglomeration, uptake, and interaction using a high illuminating system. Int. J. Tox.26, 135–141 (2007). ArticleCASGoogle Scholar
- Schrand, A.M. et al. Are diamond nanoparticles cytotoxic? J. Phys. Chem. B.111, 2–7 (2007a). ArticleCASPubMedGoogle Scholar
- Schrand, A.M., Dai, L., Schlager, J.J., Hussain, S.M. & Osawa, E. Differential biocompatibility of carbon nanotubes and nanodiamonds. Diam. Relat. Mater.16, 2118–2123 (2007b). ArticleCASGoogle Scholar
- Schrand, A.M. et al. Interaction and biocompatibility of multi-walled carbon nanotubes in PC-12 cells. Int. J. Neuroprot. Neuroregener.3, 115–121 (2007c). CASGoogle Scholar
- Wagner, A.J. et al. Cellular interaction of different forms of aluminum nanoparticles in rat alveolar macrophages. J. Phys. Chem. B.111, 7353–7359 (2007). ArticleCASPubMedGoogle Scholar
- Schrand, A.M., Braydich-Stolle, L.K., Schlager, J.J., Dai, L. & Hussain, S.M. Can silver nanoparticles be useful as potential biological labels? Nanotechnology19, 1–13 (2008a). ArticleCASGoogle Scholar
- Carlson, C. et al. Uniques cellular interaction of silver nanoparticles: size-dependent generation of reactive oxygen species. J. Phys. Chem. B.112, 13608–13619 (2008). ArticleCASPubMedGoogle Scholar
- Murdock, R.C., Braydich-Stolle, L., Schrand, A.M., Schlager, J.J. & Hussain, S.M. Characterization of nanomaterial dispersion in solution prior to in vitro exposure using dynamic light scattering technique. Tox. Sci.101, 239–253 (2008). ArticleCASGoogle Scholar
- Braydich-Stolle, L.K. et al. Crystal structure mediates mode of cell death in TiO2 nanotoxicity. J. Nanopart. Res.11, 1361–1374 (2008). ArticleCASGoogle Scholar
- Yu, K.O. et al. Toxicity of amorphous silica nanoparticles in mouse keratinocytes. J. Nanopart. Res.11, 15–24 (2009). ArticleCASGoogle Scholar
- Hussain, S.M. et al. Toxicity evaluation for safe use of nanomaterials: recent achievements and technical challenges. Adv. Mat.21, 1–11 (2009). ArticleCASGoogle Scholar
- Schrand, A.M., Citan, S.A. & Shenderova, O.A. Nanodiamond particles: properties and perspectives for bioapplications. Crit. Rev. Solid State Mater. Sci.34, 18–74 (2009). ArticleCASGoogle Scholar
- Colvin, V. The potential environmental impact of engineered nanomaterials. Nat. Biotechnol.21, 1166–1170 (2003). ArticleCASPubMedGoogle Scholar
- Nel, A.E. et al. Understanding biophysicochemical interactions at the nano-bio interface. Nat. Mater.8, 543–557 (2009). ArticleCASPubMedGoogle Scholar
- Ryman-Rasmussen, J.P., Riviere, J.E. & Monteiro-Rivere, N.A. Surface coatings determine cytotoxicity and irritation potential of quantum dot nanoparticles in epidermal keratinocytes. Soc. Invest. Derm.127, 143–153 (2006). ArticleCASGoogle Scholar
- Zhang, L.W., Zeng, L., Barron, A.R. & Monteiro-Riviere, N.A. Biological interactions of quantum dot nanoparticles in skin and in human epidermal keratinocytes. Tox. Appl. Pharm.228, 200–211 (2008). ArticleCASGoogle Scholar
- Zhang, L.W. & Monteiro-Riviere, N.A. Mechanisms of quantum dot nanoparticle cellular uptake. Tox. Sci.110, 138–155 (2009). ArticleCASGoogle Scholar
- de Jonge, N., Peckys, D.B., Kremers, G.J. & Pistona, D.W. Electron microscopy of whole cells in liquid with nanometer resolution. Proc. Natl. Acad. Sci. USA106, 2159–2164 (2009). ArticlePubMedPubMed CentralGoogle Scholar
- Mayhew, T.M., Mühlfeld, C., Vanhecke, D. & Ochs, M. A review of recent methods for efficiently quantifying immunogold and other nanoparticles using TEM sections through cells, tissues and organs. Ann. Anat.191, 153–170 (2009). ArticleCASPubMedGoogle Scholar
- Allen, T.D. et al. Visualization of the nucleus and nuclear envelope in situ by SEM in tissue culture cells. Nat. Protoc.2, 1180–1184 (2007). ArticleCASPubMedGoogle Scholar
- Allen, T.D. et al. A protocol for isolating Xenopus oocyte nuclear envelope for visualization and characterization by scanning electron microscopy (SEM) or transmission electron microscopy (TEM). Nat. Protoc.2, 1166–1172 (2007). ArticleCASPubMedGoogle Scholar
- Allen, T.D. et al. Generation of cell-free extracts of Xenopus eggs and demembranated sperm chromatin for the assembly and isolation of in vitro–formed nuclei for western blotting and scanning electron microscopy (SEM). Nat. Protoc.2, 1173–1179 (2007). ArticleCASPubMedGoogle Scholar
- Wilhelm, C., Gazeau, F., Roger, J., Pons, J.N. & Bacri, J.C. Interaction of anionic superparamagnetic nanoparticles with cells: kinetic analyses of membrane adsorption and subsequent internalization. Langmuir18, 8148–8155 (2002). ArticleCASGoogle Scholar
- Conner, S.D. & Schmid, S.L. Regulated portals of entry into the cell. Nature422, 37–44 (2003). ArticleCASPubMedGoogle Scholar
- Shukla, R. et al. Biocompatibility of gold nanoparticles and their endocytotic fate inside the cellular compartment: a microscopic overview. Langmuir21, 10644–10654 (2005). ArticleCASPubMedGoogle Scholar
- Kam, N.W.S., Liu, Z. & Dai, H. Carbon nanotubes as intracellular transporters for proteins and DNA: an investigation of the uptake mechanism and pathway. Angew. Chem. Int. Ed.45, 577–581 (2006). ArticleCASGoogle Scholar
- Dobrovolskaia, M.A. & McNeil, S.E. Immunological properties of engineered nanomaterials. Nat. Nanotech.2, 469–478 (2007). ArticleCASGoogle Scholar
- Verma, A. et al. Surface-structure-regulated cell-membrane penetration by monolayer-protected nanoparticles. Nat. Mater.7, 588–595 (2008). ArticleCASPubMedPubMed CentralGoogle Scholar
- Jin, H., Heller, D.A. & Strano, M.S. Single-particle tracking of endocytosis and exocytosis of single-walled carbon nanotubes in HIH3T3 cells. Nano Lett.8, 1577–1585 (2008). ArticlePubMedGoogle Scholar
- Yu, J. et al. Effect of surface functionality of magnetic silica nanoparticles on the cellular uptake by Glioma cells in vitro. J. Mater. Chem.19, 1265–1270 (2009). ArticleCASGoogle Scholar
- Geiser, M. et al. Ultrafine particles cross cellular membranes by nonphagocytic mechanisms in lungs and in cultured cells. Environ. Health Perspect.113, 1555–1560 (2005). ArticlePubMedPubMed CentralGoogle Scholar
- Porter, A.E. et al. Visualizing the uptake of C60 to the cytoplasm and nucleus of human monocyte-derived macrophage cells using energy-filtered transmission electron microscopy and electron tomography. Enivron. Sci. Technol.41, 3012–3017 (2007). ArticleCASGoogle Scholar
- Porter, A.E. et al. Direct imaging of single-walled carbon nanotubes in cells. Nat. Nanotechnol.2, 713–717 (2007). ArticleCASPubMedGoogle Scholar
- Cheng, C. et al. Toxicity and imaging of multi-walled carbon nanotubes in human macrophage cells. Biomaterials30, 4152–4160 (2009). ArticleCASPubMedGoogle Scholar
- Steinbrecht, R.A. Freeze-substitution and freeze-drying. in Cryotechniques in Biological Electron Microscopy (eds. Steinbrecht, R.A. & Zierold, K.) 149–172 (K. Springer-Verlag, Berlin, 1987).
- Parthasarathy, M.V. Chapter 5 freeze-substitution. Methods Cell Biol.49, 57–69 (1995). ArticleCASPubMedGoogle Scholar
- Lucic, V. et al. Multiscale imaging of neurons grown in culture: from light microscopy to cryoelectron tomography. J. Struct. Biol.160, 146–156 (2007). ArticlePubMedGoogle Scholar
- Satori, A. et al. Correlative microscopy: bridging the gap between fluorescence light microscopy and cryoelectron tomography. J. Struct. Biol.160, 135–145 (2007). ArticleGoogle Scholar
- Hess, M.W., Muller, M., Debbage, P.L., Vetterlein, M. & Pavelka, M. Cryopreparation provides new insight into the effects of brefeldin A on the structure of the HepG2 Gel apparatus. J. Struct. Biol.130, 63–72 (2000). ArticleCASPubMedGoogle Scholar
- Marko, M. Focused ion beam thinning of frozen hydrated biological specimens for cryoelectron microscopy. Nat. Methods4, 215–217 (2007). ArticleCASPubMedGoogle Scholar
- Riley, M.R. et al. Comparison of the sensitivity of three lung derived cell lines to metals from combustion derived particulate matter. Toxicol. In Vitro19, 411–419 (2005). ArticleCASPubMedGoogle Scholar
- Ricarda-Lorenz, M. et al. Uptake of functionalized, fluorescent-labeled polymeric particles in different cell lines and stem cells. Biomaterials27, 2820–2828 (2006). ArticleCASGoogle Scholar
- Sayes, C.M., Reed, K.L. & Warheit, D.B. Assessing toxicity of fine and nanoparticles: comparing in vitro measurements to in vivo pulmonary toxicity profiles. Toxicol. Sci.97, 163–180 (2007). ArticleCASPubMedGoogle Scholar
- Chang, J.-S., Chang, K.L.B., Hwang, D.-F. & Kong, K.-L. In vitro cytotoxicity of silica nanoparticles at high concentrations strongly depends on the metabolic activity type of the cell line. Environ. Sci. Technol.41, 2064–2068 (2007). ArticleCASPubMedGoogle Scholar
- Xia, T. et al. Cationic polystyreen nanosphere toxicity depends on cell-specific endocytoic and mitohcondrial injury pathways. ACS Nano2, 85–96 (2008). ArticleCASPubMedGoogle Scholar
- Lanone, S. et al. Comparative toxicity of 24 manufactured nanoparticles in human alveolar epithelial and macrophage cell lines. Part. Fiber Toxicol.6, 14–26 (2009). ArticleCASGoogle Scholar
- Nabiev, I. et al. Non-functionalized nanocrystals can exploit a cell's active transport machinery delivering them to specific nuclear and cytoplasmic compartments. Nano Lett.7, 3452–3461 (2007). ArticleCASPubMedGoogle Scholar
- Krüger, A. et al. Unusually tight aggregation in detonation nanodiamond: identification and disintegration. Carbon43, 1722–1730 (2005). ArticleCASGoogle Scholar
- Greiner, N., Phillips, D., Johnson, J. & Volk, F. Dimaonds in detonation soot. Nature333, 440–442 (1998). ArticleGoogle Scholar
- Liang, Y., Ozawa, M. & Krueger, A. A general procedure to functionalize agglomerating nanoparticles demonstrated on nanodiamond. ACS Nano3, 2288–2296 (2009). ArticleCASPubMedGoogle Scholar
- Mei, B.C., Susumu, K., Medintz, I.L. & Mattoussi, H. Polyethylene glycol-based bidentate ligands to enhance quantum dot and gold nanoparticle stability in biological media. Nat. Protoc.4, 412–423 (2009). ArticleCASPubMedGoogle Scholar
- Monteiro-Riviere, N.A., Inman, A.O., Wang, Y.Y. & Nemanich, R.J. Surfactant effects on carbon nanotube interactions with human epidermal keratinocytes. Nanomedicine1, 293–299 (2005). ArticleCASPubMedGoogle Scholar
- Limbach, L.K. et al. Oxide nanoparticle uptake in human lung fibroblasts: effects of particle size, agglomeration, and diffusion at low concentrations. Environ. Sci. Technol.39, 9370–9376 (2005). ArticleCASPubMedGoogle Scholar
- Shenderova, O. et al. Modification of detonation nanodiamonds by heat treatment in air. Diamond Relat. Mater.15, 1799 (2006). ArticleCASGoogle Scholar
- Morita, Y. et al. A facile and scalable process for size-controllable separation of nanodiamond particles as small as 4 nm. Small4, 2154–2157 (2008). ArticleCASPubMedGoogle Scholar
- Jamison, J.A. et al. Size dependent sedimentation properties of nanocrystals. ACS Nano2, 311–319 (2008). ArticleCASPubMedGoogle Scholar
- Teeguarden, J.G., Hinderliter, P.M., Orr, G., Thrall, B.D. & Pounds, J.G. Particokinetics in vitro: dosimetry considerations for in vitro nanoparticle toxicity assessments. Toxicol. Sci.95, 300–312 (2007). ArticleCASPubMedGoogle Scholar
- Jaiswal, J.K., Mattoussi, H., Mauro, J.M. & Simon, S.M. Long-term multiple color imaing of live cells using quantum dot bioconjugates. Nat. Biotechnol.21, 47–51 (2003). ArticleCASPubMedGoogle Scholar
- Monteiro-Riviere, N.A., Nemanich, R.J., Inman, A.O., Wang, Y.Y. & Riviere, J.E. Multi-walled carbon nanotube interactions with human epidermal keratinocytes. Toxicol. Lett.155, 377–384 (2005). ArticleCASPubMedGoogle Scholar
- Soto, K., Garza, K.M. & Murr, L.E. Cytotoxic effects of aggregated nanomaterials. Acta Biomater.3, 351–358 (2007). ArticleCASPubMedGoogle Scholar
- Warheit, D.B., Webb, T.R., Sayes, C.M., Colvin, V.L. & Reed, K.L. Pulmonary instillation studies with nanoscale TiO2 rods and dots in rats: toxicity is not dependent upon particle size and surface area. Toxicol. Sci.91, 227–236 (2006). ArticleCASPubMedGoogle Scholar
- Stoeger, T. et al. Instillation of six different ultrafine carbon particles indicates a surface area threshold dose for acute lung inflammation in mice. Environ. Health Perspect.114, 328–333 (2006). ArticlePubMedGoogle Scholar
- Elder, A. et al. Effects of subchronically inhaled carbon black in three species. I. Retention kinetics, lung inflammation, and histopathology. Toxicol. Sci.88, 614–629 (2005). ArticleCASPubMedGoogle Scholar
- Brown, D.M., Wilson, M.R., MacNee, W., Stone, V. & Donaldson, K. Size dependent proinflammatory effects of ultrafine polystyrene particles: a role for surface area and oxidative stress in the enhanced activity of ultrafines. Toxicol. Appl. Pharmacol.175, 191–199 (2001). ArticleCASPubMedGoogle Scholar
- Chithrani, B.D. & Chan, W.C.W. Elucidating the mechanisms of cellular uptake and removal of protein-coated gold nanoparticles of different sizes and shapes. Nano Lett.7, 1542–1550 (2007). ArticleCASPubMedGoogle Scholar
- Nativo, P., Prior, I.A. & Brust, M. Uptake and intracellular fate of surface-modified gold nanoparticles. ACS Nano2, 1639–1644 (2008). ArticleCASPubMedGoogle Scholar
- Gopee, N.V. et al. Migration of intradermally injected quantum dots to sentinel organs in mice. Toxicol. Sci.98, 249–257 (2007). ArticleCASPubMedGoogle Scholar
- Gojova, A. et al. Induction of inflammation in vascular endothelial cells by metal oxide nanoparticles: effect of particle composition. Environ. Health Perspect.115, 403–409 (2007). ArticleCASPubMedGoogle Scholar
- Gratton, S.E. et al. The effect of particle design on cellular internalization pathways. Proc. Natl. Acad. Sci. USA105, 11613–11618 (2008). ArticlePubMedPubMed CentralGoogle Scholar
- Vincent, A. et al. Protonated nanoparticle surface governing ligand tethering and cellular targeting. ACS Nano3, 1203–1211 (2009). ArticleCASPubMedPubMed CentralGoogle Scholar
- Karnovsky, M.J. A formaldehyde-glutaraldehyde fixative of high osmolality for use in electron microscopy. JCB27, 137A–138A (1965). Google Scholar
- McDowell, E.M. & Trump, B.F. Histologic fixatives suitable for diagnostic light and electron microscopy. Arch. Pathol. Lab. Med.100, 405–414 (1976). CASPubMedGoogle Scholar
- Monteiro-Riviere, N. & Inman, A. Challenges for assessing carbon nanomaterial toxicity to the skin. Carbon44, 1070–1078 (2006). ArticleCASGoogle Scholar
- Rouse, J.G., Yang, J., Barron, A.R. & Monteiro-Riviere, N.A. Fullerene-based amino acid nanoparticle interactions with human epidermal keratinocytes. Toxicol. In Vitro20, 1313–1320 (2006). ArticleCASPubMedGoogle Scholar
- Zhang, L.W., Zeng, L., Barron, A.R. & Monteiro-Riviere, N.A. Biological interactions of functionalized single-wall carbon nanotubes in human epidermal keratinocytes. Int. J. Toxicol.26, 103–113 (2007). ArticleCASPubMedGoogle Scholar
- Zhang, Y. & Zhang, J. Surface modification of monodisperse magnetite nanoparticles for improved intracellular uptake to breast cancer cells. J. Colloid Interface Sci.283, 352–357 (2005). ArticleCASPubMedGoogle Scholar
- Millonig, G. Advantages of a phosphate buffer for osmium tetroxide solutions in fixation. J. Appl. Phys.32, 1637 (1961). Google Scholar
- Reynolds, E.S. Use of lead citrate at high pH as an electron-opaque stain in electron microscopy. J. Cell Biol.17, 208–212 (1963). ArticleCASPubMedPubMed CentralGoogle Scholar
- Graham, L. & Orenstein, J.M. Processing tissue and cells for transmission electron microscopy in diagnostic pathology and research. Nat. Protoc.2, 2439–2450 (2007). ArticleCASPubMedPubMed CentralGoogle Scholar
- Robards, A.W. & Wilson, A.J. Procedure in Electron Microscopy: Module 5:5 Basic Biological Preparation Techniques for TEM 5:5.1–5:5.28 (John Wiley & Son, Hoboken, NJ, 1999).
- Aderem, A. & Underhill, D.M. Mechanisms of phagocytosis in macrophages. Annu. Rev. Immunol.17, 593–623 (1999). ArticleCASPubMedGoogle Scholar
Acknowledgements
A.M.S. received funding from the National Research Council (NRC) Fellowship program funded by the Joint Science and Technology Office for Chemical and Biological Defense (JSTO-CBD), a program administered by the Defense Threat Reduction Agency (DTRA).